Review
Rev Diabet Stud,
2010,
7(2):105-111 |
DOI 10.1900/RDS.2010.7.105 |
Pancreatic Stem/Progenitor Cells for the Treatment of Diabetes
Hirofumi Noguchi1,2
1Regenerative Research Islet Transplant Program, Baylor Research Institute, 1400 8th Avenue, Fort Worth, TX 76104, USA
2Institute of Biomedical Studies, Baylor University, Waco, TX 76798, USA
Manuscript submitted June 23, 2010; resubmitted July 20, 2010; accepted July 22, 2010.
Keywords: diabetes, pancreatic stem/progenitor cells, duct, pancreatic endoderm, beta-cell, islet, acinar, islet transplantation
Abstract
Patients with type 1 diabetes, and most patients with type 2 diabetes, have associated hyperglycemia due to the absence or reduction of insulin production by pancreatic β-cells. Surgical resection of the pancreas may also cause insulin-dependent diabetes depending on the size of the remaining pancreas. Insulin therapy has greatly improved the quality of life of diabetic patients, but this method is inaccurate and requires lifelong treatment that only mitigates the symptoms. The successes achieved over the last few decades by the transplantation of whole pancreas and isolated islets suggest that diabetes can be cured by the replenishment of deficient β-cells. These observations are proof-of-principle and have intensified interest in treating diabetes by cell transplantation, and by the use of stem cells. Pancreatic stem/progenitor cells could be one of the sources for the treatment of diabetes. Islet neogenesis, the budding of new islets from pancreatic stem/progenitor cells located in or near pancreatic ducts, has long been assumed to be an active process in the postnatal pancreas. Several in vitro studies have shown that insulin-producing cells can be generated from adult pancreatic ductal tissues. Acinar cells may also be a potential source for differentiation into insulin-producing cells. This review describes recent progress on pancreatic stem/progenitor cell research for the treatment of diabetes.
Abbreviations: bHLH - basic helix-loop-helix; CA 19-9 - carbohydrate antigen 19-9 (glycoprotein); CA-II - carbonic anhydrase II; CD34 - cluster of differentiation 34 (surface glycoprotein, cell-cell adhesion factor, mediates attachment of stem cells to bone marrow); CD45 - cluster of differentiation 45 (signaling molecule, known as protein tyrosine phosphatase receptor type C); CD133 - cluster of differentiation 133 (surface glycoprotein, also known as Prominin 1 (PROM1), expressed by different stem and progenitor cells); CK-19 - cytokeratin 19 (expressed in epithelial cells of pancreatic ducts); Cre recombinase - type I topoisomerase (catalyzes site-specific recombination of DNA between loxP sites); CreER - inducible Cre recombinase (fusion protein to induce recombinase activity); Cre/loxP - recombination system to delete DNA sequences in living organisms; GFP - green fluorescent protein; GLP-1- glucagon-like peptide 1; Ipf1 - insulin promoter factor 1 (also known as Pdx1); LacZ - structural gene of lac-operon (codes for the enzyme β-galactosidase, splits lactose into galactose und glucose); LoxP - locus of crossover in P1 (can be catalyzed by Cre); MafA - v-maf musculoaponeurotic fibrosarcoma oncogene homolog A; mTert - mouse telomerase reverse transcriptase; NeuroD - neurogenic differentiation (also known as BETA2; bHLH transcription factor expressed in pancreatic cells); Ngn3 - neurogenin 3 (member of the bHLH family of transcription factors expressed in the nervous system); Pdx1 - pancreatic and duodenal homeobox 1 (transcription factor necessary for pancreas development); PP cell - pancreatic polypeptide producing cell; Ptfa1 - pancreas-specific transcription factor 1a; ROSA26 - gene locus in mice (commonly used to knock-in cDNA constructs for ubiquitous or conditional gene expression in transgenic mice); Ter199 - γ-glutamyl-(S)-(benzyl)cysteinyl-(R)-(-)-phenylglycine diethyl ester (inhibitor of glutathione S-transferases)
Introduction
Diabetes mellitus afflicts more than 200 million people worldwide. One of the primary effects is the reduction in β-cell mass. This is prevalent in all patients with type 1 diabetes, most patients with type 2 diabetes, and those with surgical diabetes (after pancreas resection). These metabolic disorders may result in the occurrence of severe systemic complications including diabetic neuropathy, nephropathy, retinopathy, heart diseases, and stroke during progression of the disease. The current therapy, using exogenous insulin supply, has greatly improved the quality of life for diabetic patients, especially type 1 diabetic patients. However, the method is inaccurate and does not completely control the minute-to-minute fluctuations in systemic blood glucose.
As an alternative therapy, clinical studies have shown that pancreas, or islet, transplantation can support glucose homeostasis in type 1 diabetics, whose β-cells have been destroyed by an autoimmune reaction [1-6]. The advantages of islet transplantation, compared with traditional pancreas or pancreas-kidney transplantation, are the minimally invasive kind of intervention and fewer complications. However, limitations include the short supply of donor pancreata, the paucity of experienced islet isolation teams, side effects of immunosuppressants, and poor long-term results [6]. These limitations have led to a search for other sources of β-cells. Interest has gained in the differentiation of progenitor cells, and in the question whether they can be directed, or "programmed" efficiently. These programming efforts are based on present understanding of how β-cells are normally generated in the embryo, and how they arise during regeneration in adults, in response to tissue damage, and disease.
This article reviews recent progress in research on pancreatic stem/progenitor cells and their differentiation into insulin-producing cells. The in vivo stimulation of these pancreatic stem/progenitor cells, or transplantation of their ex vivo expanded differentiated progenies, could represent potential strategies for diabetes treatment.
Pancreas development and key transcription factors
The vertebrate pancreas has its embryological origin in two endodermal buds that develop on the dorsal and ventral side of the duodenum [7-9]. The dorsal bud grows just below the notochord, while the ventral bud develops adjacent to the hepatic diverticulum [10]. These two pancreatic buds grow, branch, and fuse, to form the definitive pancreas composed of exocrine and endocrine cells (Figure 1). During development, cells in the pancreatic anlage migrate from the ducts, while differentiating to form clusters that eventually become islets [11]. The exocrine pancreas is a lobulated branched tissue, which includes acinar and ductal cells secreting and transporting digestive enzymes into the duodenum. The endocrine cells are grouped into islets of Langerhans, composed of five principal cell types, α, β, δ, ε, and PP, respectively secreting glucagon, insulin, somatostatin, ghrelin, and pancreatic polypeptide hormones into the bloodstream.
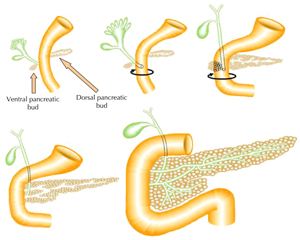 |
 |
Figure 1. Schematic representation of pancreas development. The embryonic pancreas in vertebrates forms from a dorsal and ventral protrusion of the primitive gut epithelium. These two pancreatic buds grow, branch, and fuse to form the definitive pancreas. |
|
Several classes of transcription factors are involved in the specification and differentiation of both endocrine and exocrine lineages. The newly specified pancreatic endoderm is initially marked by the expression of the pancreatic and duodenal homeobox gene 1 (Pdx1; also known as Ipf1), and then by the pancreas-specific transcription factor 1a (Ptf1a) [12, 13]. Both transcription factors are critical for pancreatic development. Pdx1 is expressed at the earliest stages in the dorsal and ventral pancreatic buds. as well as in the duodenum [14]. At later stages, it is highly expressed in β-cells, with lower levels also found in acinar cells and all rostral duodenal cells [15]. Pdx1 levels help to control the balance between endocrine and exocrine (acinar and duct) progenitors which differentiate within the pancreas [16]. Mice lacking Pdx1 do not develop a pancreas [17, 18], and mutations in the human homologue are associated with pancreatic agenesis [19]. Ptf1a is a basic helix-loop-helix (bHLH) gene that is expressed in early pancreatic progenitors (dorsal and ventral buds) [20]. In adults, it is only expressed in acinar cells [21]. Loss-of-function studies in mice have demonstrated that Ptf1a is essential for acinar cell development, and plays an important role in endocrine cell development as well [20, 22]. In humans, PTF1A gene mutations are associated with pancreatic and cerebellar agenesis [23].
Notch signaling also helps to regulate the balance of exocrine and endocrine cells, probably by allowing the expansion of an undifferentiated pancreatic progenitor population [24-26]. Loss of Notch signaling allows the endocrine lineage to develop, which requires, and is marked by, the bHLH transcription factor, neurogenin 3 (Ngn3) [12, 24, 27]. Mice lacking Ngn3 do not develop endocrine cells, and exhibit disordered acinar polarity. Ngn3 directly influences the expression of another islet-specific bHLH gene, neurogenic differentiation (NeuroD; also known as BETA2) [28]. A loss of NeuroD/BETA2 function implicates a phenotype similar to Ngn3, but with less severity. This phenotype leads to a diminished number of all endocrine cell types [29], and definitive β-cells are generated under the influence of the v-mafmusculoaponeuroticfibrosarcoma oncogene homolog A (MafA) transcription factor [30, 31].
Tissue-specific stem/progenitor cells in the pancreas
The existence of true pancreatic "stem" cells is still controversial. At present, common wisdom considers that the bulk of normal islet renewal and pancreas regeneration following damage (such as 90% pancreatectomy and partial duct ligation), is achieved by β-cell replication [32] and/or differentiation of pancreatic (NGN-3-expressing) progenitor cells located near or within ducts [33]. In fact, putative pancreatic stem cells have been isolated from adult rodent pancreas. In addition to others, we have shown that isolated mouse pancreatic stem cells are capable of self-renew and mulipotency [34, 35]. These cells were isolated from a duct-rich population, and expressed PDX1 transcription factor and cytokeratin (CK)-19. The cells differentiated into insulin-, glucagon-, and somatostatin-producing cells [34, 35]. These two studies demonstrated the possibility of multipotent stem cells located within or near adult pancreatic ducts. On the other hand, human cells from the duct-rich population were unable to divide after 30 days, even under several culture conditions. However, they were able to differentiate into insulin-producing cells [36]. There are some differences in the methodologies used in the two studies, e.g. culture conditions, isolation stress, and differences in the species.
In our study, human pancreatic stem cells were unable to maintain an undifferentiated state under any of the selected culture conditions. Given that the culture conditions for embryonic stem cells are different between human and mouse, we consider that further evaluation of other culture conditions needs to be carried out. It is possible that pancreas preservation and/or isolation stress affect the survival of human pancreatic stem cells, because human islet isolation is more stressful for cells than mouse islet isolation. Another possibility is that the mouse pancreas contain stem cells, whereas the human pancreas does not. Although many researchers have shown that there are pancreatic "progenitor" cells in the human pancreas which have multipotent capacity to differentiate into insulin-, glucagon-, and somatostatin-producing cells, no one has shown so far whether there are human pancreatic "stem" cells with multipotent and self-renew capacity.
The identification and study of tissue stem/progenitor cells has been facilitated by the generation of transgenic mouse models such as the mouse telomerase reverse transcriptase (mTert)-driven green fluorescent protein (GFP) mouse [37]. GFP-positive cells were found in the non-islet pancreatic tissue after treatment for 3 days with exendin-4, the analog of glucagon-like peptide 1 (GLP-1) [38]. This finding suggests the presence of activated stem cells in the mouse pancreas.
The advantage of tissue-specific stem/progenitor cells is their attribution to a specific differentiation pathway, which may require less in vitro manipulation to obtain fully functional β-cells than the use of less committed pluripotent stem cells. β-cells have a limited ability to expand, especially in the adult mouse and human, such that their restricted growth ability does not permit recovery from cell loss in type 1 diabetes [39]. Therefore, it is important to identify pancreatic stem/progenitor cells, especially "stem" cells which have self-renew capacity to yield a sufficient number of β-cells for the treatment of diabetes.
Pancreatic duct cells
During embryonic development, pancreatic cells migrate from the ducts, differentiate to form clusters, and finally become islets [11]. Therefore, the postnatal pancreatic duct may be the main source of progenitors for pancreatic growth and regeneration. Several in vitro studies have shown that insulin-producing cells can be generated from adult pancreatic ductal tissues [40-46]. Human adult ductal tissues cultured with matrigel formed islet-like buds, and differentiated into endocrine cells [41, 42]. Moreover, ductal cells purified from dispersed islet-depleted human pancreatic tissue using CA19-9 antibody differentiated into insulin-producing cells [46]. Other groups showed the isolation of multipotent pancreatic progenitors from both neonatal and adult pancreata using fluorescent activated cell sorting [44, 45]. The cells purified with CD133 (+), CD34 (-), CD45 (-), Ter199 (-) expressed the duct marker, CK-19 and could differentiate into pancreatic endocrine and acinar cells. It was also shown that GFP-negative pancreatic tissue from transgenic mice expresses GFP under the control of the mouse insulin promoter. When this tissue was cultured for 1 week, 1-3% of the cells expressed GFP [47].
Inada et al. generated transgenic mice expressing human carbonic anhydrase II (CAII) promoter-Cre recombinase, or the inducible form CreER, to cross with ROSA26 loxP-Stop-loxP LacZ reporter mice [48]. These genetic lineage-tracing experiments showed that within the pancreas, CA-II-expressing cells act as progenitors giving rise to new islets and acini, which emerge normally after birth and after injury (ductal ligation). Another study showed activation of NGN-3-expressing cells located near or within ducts. These cells contributed to new β-cell formation after partial duct ligation in adult mice [33]. Hence, there are clear indications that duct cells can act as pancreatic progenitors in adult rodents.
The Edmonton group attempted to define a correlation between graft cellular composition and long-term transplant success, using eighty-three transplanted human islet grafts [49, 50]. A significant positive correlation was observed between the number of ductal-epithelial cells transplanted, and long-term metabolic success, as assessed by an intravenous glucose tolerance test at approximately two years post-transplantation. Whereas, no significant correlation was observed between the total islet equivalents and long-term metabolic success. This data showed that the presence of ductal cells in clinical islet transplantation may improve the long-term metabolic outcome.
Pancreatic acinar cells
The transdifferentiation of acinar cells into islets has also been proposed [51-53]. Initial studies showed that acinar cells from human pancreas were able to transdifferentiate into pancreatic duct cells expressing CK-19 [54]. Baeyens et al. showed that manipulations of cell culture conditions have yielded functional β-cells from rat exocrine cells with a combination of EGF and leukemia inhibitory factor [52]. Minami et al. showed that pancreatic acinar cells could transdifferentiate into insulin-secreting cells with secretory properties similar to those of native pancreatic β-cells [53]. The frequency of insulin-positive cells was only 0.01% in the initial preparation and increased to approximately 5% under culture conditions. Analysis by the Cre/loxP-based direct cell lineage-tracing system indicates that these new cells originate from amylase/elastase-expressing pancreatic acinar cells.
Melton’s group showed in vivo reprogramming of adult pancreatic exocrine cells to yield β-cells [55]. Using a strategy of re-expressing key developmental regulators in vivo through adenoviral delivery, they identified a specific combination of three transcription factors (Pdx1, Ngn3, and MafA) that reprogrammed the differentiated pancreatic exocrine cells in adult mice into cells that closely resemble β-cells. The induced β-cells were able to ameliorate hyperglycemia by remodeling local vasculature and secreting insulin. Interestingly, the direct shift from acinar to β-cells did not involve activation of the cell cycle or dedifferentiation. For this reason, the forced reprogramming of exocrine cells cannot be regarded as evidence for the potential of progenitor cells under unmanipulated conditions. This approach might have lower risk of tumor formation than one that involves a self-renewable, pluripotent cell type. However, the use of virus vectors is a disadvantage of this study.
Other stem cells and future prospects
The generation of β-cells may be accomplished through the differentiation of stem/progenitor cells derived from endoderm in other organs such as liver and gastrointestinal tract [56-66], embryonic stem (ES) cells [67, 68], and induced pluripotent stem (iPS) cells [69] (Figure 2). Recent progress in the search for new sources of β-cells has opened up several possibilities for the development of new treatments for diabetes. The issue of how stem/progenitor cells could be induced to yield fully functional islets is unresolved so far. We need further investigations to establish cell-based therapies for the potential treatment and cure of diabetes.
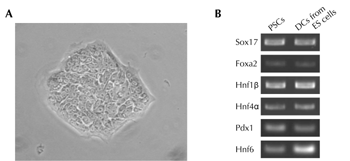 |
 |
Figure 2. Characterization of mouse pancreatic stem cells. A. Morphology of mouse pancreatic stem cells. B. RT-PCR analysis of endodermal/pancreatic cell marker genes in mouse pancreatic stem cells. Mouse pancreatic stem cells (PSCs) were analyzed by RT-PCR. Differentiated cells (DCs) derived from embryonic stem (ES) cells by stepwise protocol [67] were used as a positive control. |
|
Disclosures (conflict of interests statement): The author reports no conflict of interests.
Acknowledgments:
The author wishes to thank Dr. Steven Phillips for editing the manuscript.
References
- Shapiro AM, Lakey JR, Ryan EA, Korbutt GS, Toth E, Warnock GL, Kneteman NM, Rajotte RV. Islet transplantation in seven patients with type 1 diabetes mellitus using a glucocorticoid-free immunosuppressive regimen. N Engl J Med 2000. 343:230-238. [DOD] [CrossRef]
- Noguchi H, Iwanaga Y, Okitsu T, Nagata H, Yonekawa Y, Matsumoto S. Evaluation of islet transplantation from non-heart beating donors. Am J Transplant 2006. 6:2476-2482. [DOD] [CrossRef]
- Noguchi H, Ikemoto T, Naziruddin B, Jackson A, Shimoda M, Fujita Y, Chujo D, Takita M, Kobayashi N, Onaca N, Levy MF, Matsumoto S. Iodixanol-controlled density gradient during islet purification improves recovery rate in human islet isolation. Transplantation 2009. 87:1629-1635. [DOD] [CrossRef]
- Ricordi C, Strom TB. Clinical islet transplantation: Advances and immunological challenges. Nat Rev Immuunol 2004. 4:259-268. [DOD] [CrossRef]
- Hering BJ, Kandaswamy R, Ansite JD, Eckman PM, Nakano M, Sawada T, Matsumoto I, Ihm SH, Zhang HJ, Parkey J, Hunter DW, Sutherland DE. Single-donor, marginal-dose islet transplantation in patients with type 1 diabetes. JAMA 2005. 293:830-835. [DOD] [CrossRef]
- Robertson RP. Islet transplantation as a treatment for diabetes - a work in progress. N Engl J Med 2004. 350:694-705. [DOD] [CrossRef]
- Edlund H. Pancreatic organogenesis - developmental mechanisms and implications for therapy. Nat Rev Genet 2002. 3:524-532. [DOD] [CrossRef]
- Zaret KS, Grompe M. Generation and regeneration of cells of the liver and pancreas. Science 2008. 322:1490-1494. [DOD] [CrossRef]
- Zaret KS. Genetic programming of liver and pancreas progenitors: lessons for stem-cell differentiation. Nat Rev Genet 2008. 9:329-340. [DOD] [CrossRef]
- Slack JM. Developmental biology of the pancreas. Development 1995. 121:1569-1580. [DOD]
- Gu G, Brown JR, Melton DA. Direct lineage tracing reveals the ontogeny of pancreatic cell fates during mouse embryogenesis. Mech Dev 2003. 120:35-43. [DOD] [CrossRef]
- Gu G, Dubauskaite J, Melton DA. Direct evidence for the pancreatic lineage: NGN3+ cells are islet progenitors and are distinct from duct progenitors. Development 2002. 129:2447-2457. [DOD]
- Kawaguchi Y, Cooper B, Gannon M, Ray M, MacDonald RJ, Wright CV. The role of the transcriptional regulator Ptf1a in converting intestinal to pancreatic progenitors. Nat Genet 2002. 32:128-134. [DOD] [CrossRef]
- Wright CV, Schnegelsberg P, De Robertis EM. XlHbox 8: a novel Xenopushomeo protein restricted to a narrow band of endoderm. Development 1989. 105:787-794. [DOD]
- Jonsson J, Ahlgren U, Edlund T, Edlund H. IPF1, a homeodomain protein with a dual function in pancreas development. Int J Dev Biol 1995. 39:789-798. [DOD]
- Fujitani Y, Fujitani S, Boyer DF, Gannon M, Kawaguchi Y, Ray M, Shiota M, Stein RW, Magnuson MA, Wright CV. Targeted deletion of a cis-regulatory region reveals differential gene dosage requirements for Pdx1 in foregut organ differentiation and pancreas formation. Genes Dev 2006. 20:253-266. [DOD] [CrossRef]
- Jonsson J, Carlsson L, Edlund T, Edlund H. Insulin-promoter-factor 1 is required for pancreas development in mice. Nature 1994. 371:606-609. [DOD] [CrossRef]
- Offield MF, Jetton TL, Labosky PA, Ray M, Stein RW, Magnuson MA, Hogan BL, Wright CV. PDX-1 is required for pancreatic outgrowth and differentiation of the rostral duodenum. Development 1996. 122:983-995. [DOD]
- Stoffers DA, Zinkin NT, Stanojevic V, Clarke WL, Habener JF. Pancreatic agenesis attributable to a single nucleotide deletion in the human IPF1 gene coding sequence. Nat Genet 1997. 15:106-110. [DOD] [CrossRef]
- Kawaguchi Y, Cooper B, Gannon M, Ray M, MacDonald RJ, Wright CV. The role of the transcriptional regulator Ptf1a in converting intestinal to pancreatic progenitors. Nat Genet 2002. 32:128-134. [DOD] [CrossRef]
- Krapp A, Knöfler M, Frutiger S, Hughes GJ, Hagenbüchle O, Wellauer PK. The p48 DNA-binding subunit of transcription factor PTF1 is a new exocrine pancreas-specific basic helix-loop-helix protein. EMBO J 1996. 15:4317-4329. [DOD]
- Krapp A, Knöfler M, Ledermann B, Bürki K, Berney C, Zoerkler N, Hagenbüchle O, Wellauer PK. The bHLH protein PTF1-p48 is essential for the formation of the exocrine and the correct spatial organization of the endocrine pancreas. Genes Dev 1998. 12:3752-3763. [DOD] [CrossRef]
- Sellick GS, Barker KT, Stolte-Dijkstra I, Fleischmann C, Coleman RJ, Garrett C, Gloyn AL, Edghill EL, Hattersley AT, Wellauer PK, Goodwin G, Houlston RS. Mutations in PTF1A cause pancreatic and cerebellar agenesis. Nat Genet 2004. 36:1301-1305. [DOD] [CrossRef]
- Apelqvist A, Li H, Sommer L, Beatus P, Anderson DJ, Honjo T, Hrabe de Angelis M, Lendahl U, Edlund H. Notch signalling controls pancreatic cell differentiation. Nature 1999. 400:877-8781. [DOD] [CrossRef]
- Jensen J, Pedersen EE, Galante P, Hald J, Heller RS, Ishibashi M, Kageyama R, Guillemot F, Serup P, Madsen OD. Control of endodermal endocrine development by Hes-1. Nat Genet 2000. 24:36-44. [DOD] [CrossRef]
- Nakhai H, Siveke JT, Klein B, Mendoza-Torres L, Mazur PK, Algül H, Radtke F, Strobl L, Zimber-Strobl U, Schmid RM. Conditional ablation of Notch signaling in pancreatic development. Development 2008. 135:2757-2765. [DOD] [CrossRef]
- Gradwohl G, Dierich A, LeMeur M, Guillemot F. neurogenin3 is required for the development of the four endocrine cell lineages of the pancreas. Proc Natl Acad Sci U S A 2000. 97:1607-1611. [DOD] [CrossRef]
- Huang HP, Liu M, El-Hodiri HM, Chu K, Jamrich M, Tsai MJ. Regulation of the pancreatic islet-specific gene BETA2 (neuroD) by neurogenin 3. Mol Cell Biol 2000. 20:3292-3307. [DOD] [CrossRef]
- Naya FJ, Huang HP, Qiu Y, Mutoh H, DeMayo FJ, Leiter AB, Tsai MJ. Diabetes, defective pancreatic morphogenesis, and abnormal enteroendocrine differentiation in BETA2/neuroD-deficient mice. Genes Dev 1997. 11:2323-2334. [DOD] [CrossRef]
- Matsuoka TA, Zhao L, Artner I, Jarrett HW, Friedman D, Means A, Stein R. Members of the large Maf transcription family regulate insulin gene transcription in islet beta cells. Mol Cell Biol 2003. 23:6049-6062. [DOD] [CrossRef]
- Olbrot M, Rud J, Moss LG, Sharma A. Identification of beta-cell-specific insulin gene transcription factor RIPE3b1 as mammalian MafA. Proc Natl Acad Sci U S A 2002. 99:6737-6742. [DOD] [CrossRef]
- Dor Y, Brown J, Martinez OI, Melton DA. Adult pancreatic beta-cells are formed by self-duplication rather than stem-cell differentiation. Nature 2004. 429:41-46. [DOD] [CrossRef]
- Xu X, D'Hoker J, Stange G, Bonne S, De Leu N, Xiao X, Van de Casteele M, Mellitzer G, Ling Z, Pipeleers D, et al. Beta cells can be generated from endogenous progenitors in injured adult mouse pancreas. Cell 2008. 132:197-207. [DOD] [CrossRef]
- Yamamoto T, Yamato E, Taniguchi H, Shimoda M, Tashiro F, Hosoi M, Sato T, Fujii S, Miyazaki JI. Stimulation of cAMPsignalling allows isolation of clonal pancreatic precursor cells from adult mouse pancreas. Diabetologia 2006. 49:2359-2367. [DOD] [CrossRef]
- Noguchi H, Oishi K, Ueda M, Yukawa H, Hayashi S, Kobayashi N, Levy MF, Matusmoto S. Establishment of mouse pancreatic stem cell line. Cell Transplant 2009.18:563-571. [DOD]
- Noguchi H, Naziruddin B, Jackson A, Shimoda M, Ikemoto T, Fujita Y, Chujo D, Takita M, Kobayashi N, Onaca N, Hayashi S, Levy MF, Matsumoto S. Characterization of human pancreatic progenitor cells. Cell Transplant 2010. In press. [DOD]
- Breault DT, Min IM, Carlone DL, Farilla LG, Ambruzs DM, Henderson DE, Algra S, Montgomery RK, Wagers AJ, Hole N. Generation of mTert-GFP mice as a model to identify and study tissue progenitor cells. Proc Natl Acad Sci U S A 2008. 105:10420-10425. [DOD] [CrossRef]
- Aguayo-Mazzucato C, Bonner-Weir S. Stem cell therapy for type 1 diabetes mellitus. Nat Rev Endocrinol 2010. 6:139-148. [DOD] [CrossRef]
- Nishio J, Gaglia JL, Turvey SE, Campbell C, Benoist C, Mathis D. Islet recovery and reversal of murine type 1 diabetes in the absence of any infused spleen cell contribution. Science 2006. 311:1775-1778. [DOD] [CrossRef]
- Noguchi H, Kaneto H, Weir GC, Bonner-Weir S. PDX-1 protein containing its own antennapedia-like protein transduction domain can transduce pancreatic duct and islet cells. Diabetes 2003. 52:1732-1737. [DOD] [CrossRef]
- Bonner-Weir S, Taneja M, Weir GC, Tatarkiewicz K, Song KH, Sharma A, O'Neil JJ. In vitro cultivation of human islets from expanded ductal tissue. Proc Natl Acad Sci U S A 2000. 97:7999-8004. [DOD] [CrossRef]
- Gao R, Ustinov J, Pulkkinen MA, Lundin K, Korsgren O, Otonkoski T. Characterization of endocrine progenitor cells and critical factors for their differentiation in human adult pancreatic cell culture. Diabetes 2003. 52:2007-2015. [DOD] [CrossRef]
- Heremans Y, Van De Casteele M, In't Veld P, Gradwohl G, Serup P, Madsen O, Pipeleers D, Heimberg H. Recapitulation of embryonic neuroendocrine differentiation in adult human pancreatic duct cells expressing neurogenin 3. J Cell Biol 2002. 159:303-312. [DOD] [CrossRef]
- Suzuki A, Nakauchi H, Taniguchi H. Prospective isolation of multipotent pancreatic progenitors using flow-cytometric cell sorting. Diabetes 2004. 53:2143-2152. [DOD] [CrossRef]
- Oshima Y, Suzuki A, Kawashimo K, Ishikawa M, Ohkohchi N, Taniguchi H. Isolation of mouse pancreatic ductal progenitor cells expressing CD133 and c-Met by flow cytometric cell sorting. Gastroenterology 2007. 132:720-732. [DOD] [CrossRef]
- Yatoh S, Dodge R, Akashi T, Omer A, Sharma A, Weir GC, Bonner-Weir S. Differentiation of affinity-purified human pancreatic duct cells to beta-cells. Diabetes 2007. 56:1802-1809. [DOD]
- Kikugawa R, Katsuta H, Akashi T, Yatoh S, Weir GC, Sharma A, Bonner-Weir S. Differentiation of COPAS-sorted non-endocrine pancreatic cells into insulin-positive cells in the mouse. Diabetologia 2009. 52:645-652. [DOD] [CrossRef]
- Inada A, Nienaber C, Katsuta H, Fujitani Y, Levine J, Morita R, Sharma A, Bonner-Weir S. Carbonic anhydrase II-positive pancreatic cells are progenitors for both endocrine and exocrine pancreas after birth. Proc Natl Acad Sci U S A 2008. 105:19915-19919. [DOD] [CrossRef]
- Street CN, Lakey JR, Shapiro AM, Imes S, Rajotte RV, Ryan EA, Lyon JG, Kin T, Avila J, Tsujimura T, Korbutt GS. Islet graft assessment in the Edmonton Protocol: implications for predicting long-term clinical outcome. Diabetes 2004. 53(12):3107-3114. [DOD] [CrossRef]
- Street CN, Lakey JR, Rajotte RV, Shapiro AM, Kieffer TJ, Lyon JG, Kin T, Korbutt GS. Enriched human pancreatic ductal cultures obtained from selective death of acinar cells express pancreatic and duodenal homeobox gene-1 age-dependently. Rev Diabet Stud 2004. 1:66-79. [DOD] [CrossRef]
- Lardon J, Huyens N, Rooman I, Bouwens L. Exocrine cell transdifferentiation in dexamethasone-treated rat pancreas. Virchows Arch 2004. 444:61-65. [DOD] [CrossRef]
- Baeyens L, De Breuck S, Lardon J, Mfopou JK, Rooman I, Bouwens L. In vitro generation of insulin-producing beta cells from adult exocrine pancreatic cells. Diabetologia 2005. 48:49-57. [DOD] [CrossRef]
- Minami K, Okuno M, Miyawaki K, Okumachi A, Ishizaki K, Oyama K, Kawaguchi M, Ishizuka N, Iwanaga T, Seino S. Lineage tracing and characterization of insulin-secreting cells generated from adult pancreatic acinar cells. Proc Natl Acad Sci U S A 2005. 102:15116-15121. [DOD] [CrossRef]
- Hall PA, Lemoine NR. Rapid acinar to ductal transdifferentiation in cultured human exocrine pancreas. J Pathol 1992. 166:97-103. [DOD] [CrossRef]
- Zhou Q, Brown J, Kanarek A, Rajagopal J, Melton DA. In vivo reprogramming of adult pancreatic exocrine cells to beta-cells. Nature 2008. 455:627-632. [DOD] [CrossRef]
- Ferber S, Halkin A, Cohen H, Ber I, Einav Y, Goldberg I, Barshack I, Seijffers R, Kopolovic J, Kaiser N, Karasik A. Pancreatic and duodenal homeobox gene 1 induces expression of insulin genes in liver and ameliorates streptozotocin-induced hyperglycemia. Nat Med 2000. 6:568-572. [DOD] [CrossRef]
- Kojima H, Fujimiya M, Matsumura K, Younan P, Imaeda H, Maeda M, Chan L. NeuroD-betacellulin gene therapy induces islet neogenesis in the liver and reverses diabetes in mice. Nat Med 2003. 9:596-603. [DOD] [CrossRef]
- Zalzman M, Gupta S, Giri RK, Berkovich I, Sappal BS, Karnieli O, Zern MA, Fleischer N, Efrat S. Reversal of hyperglycemia in mice by using human expandable insulin-producing cells differentiated from fetal liver progenitor cells. Proc Natl Acad Sci U S A 2003. 100:7253-7258. [DOD] [CrossRef]
- Kaneto H, Nakatani Y, Miyatsuka T, Matsuoka TA, Matsuhisa M, Hori M, Yamasaki Y. PDX-1/VP16 fusion protein, together with NeuroD or Ngn3, markedly induces insulin gene transcription and ameliorates glucose tolerance. Diabetes 2005. 54:1009-1022. [DOD] [CrossRef]
- Yang L, Li S, Hatch H, Ahrens K, Cornelius JG, Petersen BE, Peck AB. In vitro trans-differentiation of adult hepatic stem cells into pancreatic endocrine hormone-producing cells. Proc Natl Acad Sci U S A 2002. 99:8078-8083. [DOD] [CrossRef]
- Nagaya M, Katsuta H, Kaneto H, Bonner-Weir S, Weir GC. Adult mouse intrahepatic biliary epithelial cells induced in vitro to become insulin-producing cells. J Endocrinol 2009. 201:37-47. [DOD] [CrossRef]
- Kojima H, Nakamura T, Fujita Y, Kishi A, Fujimiya M, Yamada S, Kudo M, Nishio Y, Maegawa H, Haneda M, et al. Combined expression of pancreatic duodenal homeobox 1 and islet factor 1 induces immature enterocytes to produce insulin. Diabetes 2002. 51:1398-1408. [DOD] [CrossRef]
- Yoshida S, Kajimoto Y, Yasuda T, Watada H, Fujitani Y, Kosaka H, Gotow T, Miyatsuka T, Umayahara Y, Yamasaki Y, Hori M. PDX-1 induces differentiation of intestinal epithelioid IEC-6 into insulin-producing cells. Diabetes 2002. 51:2505-2513. [DOD] [CrossRef]
- Suzuki A, Nakauchi H, Taniguchi H. Glucagon-like peptide 1 (1-37) converts intestinal epithelial cells into insulin-producing cells. Proc Natl Acad Sci U S A 2003. 100:5034-5039. [DOD] [CrossRef]
- Kaneto H, Miyatsuka T, Kawamori D, Matsuoka TA. Pleiotropic Roles of PDX-1 in the Pancreas. Rev Diabet Stud 2007. 4:209-225. [DOD] [CrossRef]
- Efrat S. Ex-vivo Expansion of Adult Human Pancreatic Beta-Cells. Rev Diabet Stud 2008. 5:116-122. [DOD] [CrossRef]
- D'Amour KA, Bang AG, Eliazer S, Kelly OG, Agulnick AD, Smart NG, Moorman MA, Kroon E, Carpenter MK, Baetge EE. Production of pancreatic hormone-expressing endocrine cells from human embryonic stem cells. Nat Biotechnol 2006. 24:1392-1401. [DOD] [CrossRef]
- Liew CG, Andrews PW. Stem cell therapy to treat diabetes mellitus. Rev Diabet Stud 2008. 5:203-219. [DOD] [CrossRef]
- Maehr R, Chen S, Snitow M, Ludwig T, Yagasaki L, Goland R, Leibel RL, Melton DA. Generation of pluripotent stem cells from patients with type 1 diabetes. Proc Natl Acad Sci U S A 2009. 106:15768-15773. [DOD] [CrossRef]
This article has been cited by other articles:
|
CXCR4 positive cell-derived Pdx1-high/Shh-low cells originated from embryonic stem cells improve the repair of pancreatic injury in mice
Yu T, Qing Q, Deng N, Min XH, Zhao LN, Li JY, Xia ZS, Chen QK
Cell Biol Int 2015. In press
|
|
|
Death signalobody: inducing conditional cell death in response to a specific antigen
Tone Y, Kawahara M, Kawaguchi D, Ueda H, Nagamune T
Hum Gene Ther Methods 2013. 24(3):141-150
|
|
|
Influences of the diabetes surgery on pancreatic β-cells mass
Martinez-Moreno JM, Garciacaballero M
Nutr Hosp 2013. 28(Suppl 2):88-94
|
|
|
Stem cell niches exposed to tobacco smoke
Jedrzejas M, Skowron K, Czekaj P
Przegl Lek 2012. 69(10):1063-1073
|
|
|
Protective effect of rat pancreatic progenitors cells expressing Pdx1 and nestin on islets survival and function in vitro and in vivo
Zhou SY, Zhang YS, Li Q, Zhang Y, Qi H, Zhou HX, Deng CY, Li FR
J Physiol Biochem 2012. 68(4):603-610
|
|
|
Effect of mesenchymal stem cell therapy on recovery of streptozotocin-induced diabetes mellitus in adult male albino rats: a histological and immunohistochemical study
Afifi NM
Egypt J Histol 2012. 35(3):458-469
|
|
|
Redox homeostasis in pancreatic β cells
Jezek P, Dlaskova A, Plecita-Hlavata L
Oxid Med Cell Longev 2012. 2012:932838
|
|
|
Hyperbaric oxygenation of UW solution positively impacts on the energy state of porcine pancreatic tissue
Stiegler P, Stadlbauer-Köllner V, Sereinigg M, Hackl F, Puntschart A, Schweiger M, Prenner G, Schaffellner S, Iberer F, Lackner C
Eur Surg 2011. 43(6):366-373
|
|
|
Enhancing cell therapies from the outside in: cell surface engineering using synthetic nanomaterials
Stephan MT, Irvine DJ
Nano Today 2011. 6(3):309-325
|
|
|
Stem cell therapy in diabetes mellitus: current trends
Sarath R, Rani VL
Int J Biomed Res 2011. 2(12):608-617
|
|
|
Recent progress in pancreatic islet transplantation
Kuise T, Noguchi H
World J Transplant 2011. 1(1):13-18
|
|
|